Polymeric Materials And Coronary Angioplasty Devices
By Eric R. George, Ph.D., of ERG Polymers LLC
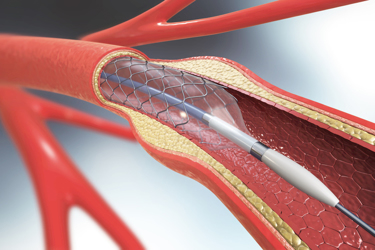
Cardiovascular diseases are responsible for over 15 million deaths worldwide per year.1 The occlusion of vessels such as the coronary or peripheral arteries hinders the flow of blood to the heart, resulting in heart attacks (myocardial infarction) and often death. Percutaneous transluminal coronary angioplasty (PTCA), a minimally invasive endovascular procedure used to widen narrowed or obstructed arteries, is enabled in many ways by polymeric materials.
In the first article in this series, “An Introduction to Polymeric Materials for Medical Devices,” I discussed polymer chemistry, key requirements for use in medical devices, and the application landscape for polymers’ successful utilization in medical devices.
This article will focus on PTCA, also known as balloon angioplasty (see references 2 -4 for a complete history of this procedure). PTCA was made possible by cardiovascular system x-ray imaging in the late 1800s.2 The first experimental evidence that eventually led to today’s modern procedures was observed by Dotter in 1964 that diagnostic catheters passing through an iliac lesion compressed the arterial plaque, leading to a larger arterial lumen. In 1974, Gruentzig developed the first double balloon catheter for the dilation of vascular stenosis.3
Polymer physics and mechanical engineering principles can contribute significantly to the understanding of balloon catheter expansion. The optimization of guide wires, guiding and balloon catheters, and stents continues to contribute to improved success rates. Their shapes, sizes, stiffness, flexibility, and internal and external lubricity are critical success factors. In this article, I discuss controlled drug release from various components and forward-looking applications such as electronic-enabled catheters and stents and bioresorbable and 3D printed stents.
Polymer Selection
We discuss in order the role of polymers in guide wires (GW), guiding catheters (GCs), balloon catheters, stents, and drug delivery. Imaged here are a guide wire and balloon catheter.
Image courtesy of Zeus.5
GWs are the first component inserted in the arterial lumens and are typically metal wires coated with lubricious, low-friction polymers such as fluoropolymers and silicones.
GCs in PTCA are typically engineered to be inserted at the femoral artery and possess all the requirements to traverse to the coronary arterial lumen. Balloon catheters and stents are delivered via the GC. The requirements include functionality as a multilayer composite, biocompatibility, and low friction/lubricity interiorly and on the surface, concomitant with mechanical fortitude for delivery of other components in its interior. Pictured here is the three-layer construction of a typical guide catheter: The inner (gray) PTFE, wire mesh, and outer jacket (blue).
Image courtesy of Zeus.5
The inner lubricity of the GC will first involve functional delivery over the GW. Lubricity is a function of a two-surface interaction. While many GC inner lumens are made from polytetrafluoroethylene (PTFE), the lowest friction option, one can tailor the GW polymeric coating (fluoropolymers, polyolefins, polyamides, silicones, etc.) to have proper and functional lubricity with a given GC inner lumen surface. The inner layer will continue to succeed with PTFE liners, but polyolefins and other polymers (including copolymers with PTFE) could exhibit low friction with superior mechanical properties to PTFE. A similar discussion can apply to the delivery of balloon catheters and stent-loaded balloon catheters.
The GC will be stiffer proximally versus distally for traversing to the arterial lumen more effectively. This can be achieved via the GC having a gradient of flexibility. For the outer layer, thermoplastic elastomers (TPE) such as polyether block amide (PEBA) and olefin-based TPE can be engineered to different moduli, strength, and surface characteristics to help achieve this functional gradient. For the proximal end, one can choose stiffer homopolymers such as engineering thermoplastics discussed in my first article. The middle mesh can be of various designs as well as materials. Metal meshes are functional, with a great track record of success with stainless steel and nickel titanium. Kevlar fibers are proving to be a solid option for meshes.
Polymer Viscoelasticity And Balloon Catheters
Balloon catheter shafts are stiffer materials made from engineering thermoplastics, polyamides (nylon), and polyesters, for example. For the inflatable segment of balloon catheters, the term “viscoelasticity” is introduced. Polymers exhibit characteristics of both viscous liquids (which permanently elongate beyond a critical stress) and elastic solids (which partially recoil after release of stress), therefore exhibiting viscoelasticity. TPE are an amalgam of softer rubber-like components (visco) and stiffer (elastic) components. When a balloon is inflated, the amount of force required to expand the balloon is proportional to the ratio and morphology of the two components. The strain is the length of the expansion. Once expanded under pressure, TPE can continue to expand (known as creep). The amount of force (stress) to maintain the expanded balloon can decrease with time (stress relaxation).
Coronary balloon materials must exhibit the capability to expand to a target diameter uniformly and precisely at the area of plaque build-up at pressures around 20 atm and maintain that diameter long enough to increase blood flow. Balloon burst pressure must exceed inflation pressure. Polymer scientists and mechanical engineers combine their skills to tailor the exact behavior of balloons for PTCA. Finite element analysis can be utilized to model the behavior of balloon catheter viscoelastic polymers.6
Current Trends In PTCA
PTCA is considered by some to be one of the most impactful contributions to human health in history. The future remains bright for even more effective coronary angioplasty. Current efforts include drug-coated balloons to inhibit restenosis,7 macromolecular approaches to prevent intimal hyperplasia after PTCA,8 recent advances in drug-eluting stents,9 and several discovery-stage programs in bioresorbable, electronic, shape memory, and 3D-printed stents.10,11
Drug-eluting balloons and stents were developed to extend the efficacy of PTCA by inhibiting restenosis, intimal hyperplasia, and thrombosis. The early drug-eluting stents exhibited mixed results, as coating a stent has its limitations. One example of coating balloon catheters with phospholipid-encapsulated anti-inflammatory, anti-restenotic pharmaceutical active ingredients and controlling the release rates is a solid option moving forward. The drugs are released during angioplasty and absorbed into the arterial lumen endothelium.7
For drug-eluting balloons (DEB), a drug-infused porous balloon is another option. Porous balloons can be prepared by expanded fluoropolymers (PTFE, for example), foamed polymers, polymer gels, and polymers with inherently large free volumes. “The porous balloon has the additional benefit that it can deliver hydrogel precursors, or even molecular coatings to the damaged surface of the vessel to form soft, antithrombotic coatings on the vessel lumen.”8
Recent advances in stents include controlled release drug-eluting stents (DES);9 bioresorbable electronic-enabled stents;10 and a potentially quintessential 3D-printed, bioresorbable, electronic, shape memory stent.11 Hu et al.9 reviewed recent progress in addressing restenosis, delayed or incomplete reendothelialization, and late-stent thrombosis. Drug release rate over time is critical to minimizing adverse events related to PTCA and the authors determined that “the factors affecting the drug release rate include the drug carrier, drug, coating methods, drug storage, elution direction, coating thickness, pore size in the coating, release conditions (release medium, pH value, temperature), and hemodynamics after the stent implantation.”
Son et al.10 “present nanomaterial designs and integration strategies for the bioresorbable electronic stent with drug-infused functionalized nanoparticles to enable flow sensing, temperature monitoring, data storage, wireless power/data transmission, inflammation suppression, localized drug delivery, and hyperthermia therapy.” Electronic stents that combine sensing of blood flow and temperature via integrated electronics could lead to increased time of efficacy for PTCA.
Yeazel et al.11 report progress toward 3D printing of bioresorbable shape memory polymer stents. 3D printing can provide the means to tailor the dimensions of stents for precise fitting to each individual patient. Bioresorbable polymer technology is advancing toward polymers that break down to be excreted without adverse events in the patient. Shape memory can enable expansion of a stent to a more precise expansion, minimizing arterial lumen mechanical damage. Shape memory approaches eliminate the need for balloon expansion deployment of stents.
Bioresorbable polymer technology continues to advance. A key limitation is that traditional bioresorbable polymers such as poly(L-lactide), poly(D-lactide), and poly(caprolactone) resorb too slowly and/or lack the prolonged mechanical properties to adequately compress the plaque of stenotic arterial lumens.
Recent advances in photochemically printed, shape memory, and/or bioresorbable polymers include poly (propylene fumarate), poly (ortho ester), and thermo-responsive poly (oligo ethylene glycol-co-vinyl acetate).11 Optimizing polymer molecular weight, degree of crystallinity and orientation (by annealing), and distribution of comonomer units will be critical factors for success aside from the chemical constitution of the polymers utilized.
Conclusion
PTCA remains a critical treatment in coronary artery disease. The procedure is multifaceted and polymers play a critical role in most components of the procedure: The first inserted guide wire is coated with lubricious polymers such as fluoropolymers, the guide catheter is basically a three-layer polymer composite consisting often of a PTFE inner lumen, a metal or liquid crystal polymer middle layer wire mesh, and the outer layer consisting of stiffer polymers like high melting polyamides on the proximal end and lower modulus polymers like thermoplastic elastomers on the distal end (poly ether-block-amides, for example). Metal stents may one day be replaced with polymers and inorganic materials that can be 3D printed and exhibit shape memory to tailor the exact dimensions before and after expansion for patient-centric improved efficacy. Electronic-enabled bioresorbable polymers that can be left in place, provide extended blood flow over current standards, and degrade and excrete with no adverse events for the patient are making progress in many R&D labs around the world.
References
- Hiob, M. A. et al., Biomaterials and Modifications in the Development of Small-Diameter Vascular Grafts, ACS Biomater. Sci. Eng., 3, 712−723 (2016).
- Wake, Ryotaro, Yoshiyama, Minoru et al., History of Coronary Angioplasty, DOI: 10.5772/22578 · Source: InTech, Chapter · November (2011).
- David P. Faxon, ed., Practical Angioplasty, Raven Press (1993).
- Lambert, B. J., Tang, F. W., and Rogers, W. J., Polymers in Medical Applications, Vol. 11, No. 7, Ch. 3, Rapra Technology LTD., (2001).
- Ridley, J., Zeus Image Library, (2021).
- F. Ibrahim, N.A. Abu Osman, J. Usman and N.A. Kadri (Eds.), Analysis of Stress on Inflation of Balloon Catheter using Finite Element Method, Biomed 06, IFMBE Proceedings 15, pp. 178-181, (2007).
- Gandhi, Pankaj J. and Murthy, Z. V. P., Investigation of Different Drug Deposition Techniques on Drug Releasing Properties of Cardiovascular Drug Coated Balloons, Ind. Eng. Chem. Res., 51, 10800−10823 (2012)
- Scott, Rebecca A. and Panitch, Alyssa, Macromolecular Approaches to Prevent Thrombosis and Intimal Hyperplasia Following Percutaneous Coronary Intervention, Biomacromolecules, 15, 2825−2832 (2014).
- Hu, Tingzhang, et al., Controlled Slow-Release Drug-Eluting Stents for the Prevention of Coronary Restenosis: Recent Progress and Future Prospects, ACS Appl. Mater. Interfaces, 7, 11695−11712 (2015).
- Son, Donghee, et al., Bioresorbable Electronic Stent Integrated with Therapeutic Nanoparticles for Endovascular Diseases, ACS Nano, vol. 9, no. 6, 5937–5946 (2015).
- Yeazel, Taylor R. and Becker, Matthew L., Advancing Toward 3D Printing of Bioresorbable Shape Memory Polymer Stents, Biomacromolecules, 21, 3957−3965 (2020).
About The Author:
Dr. Eric R. George holds a Ph.D. in polymer science and engineering from the University of Massachusetts and a B.S. in polymer science from the University of Southern Mississippi. His expertise spans engineering thermoplastics, biodegradable polymers, biomedical devices, and diseases of the eye. He is currently with ERG Polymers, LLC, and has previously held positions at GE Plastics, Zeus Inc., and Johnson & Johnson (both Johnson & Johnson Vision and Janssen Pharmaceuticals). He holds 35 U.S. patents, has published 12 refereed journal articles, and is a certified Six Sigma Black Belt. He received the 2017 Outstanding Alumnus Award from the University of Southern Mississippi College of Science and Technology.